For other uses, see Carbon cycle and Carbon sequestration. "Fixed carbon" redirects here. For the component of coal, see Coal analysis.

Biological carbon fixation, or сarbon assimilation, is the process by which living organisms convert inorganic carbon (particularly carbon dioxide, CO2) to organic compounds. These organic compounds are then used to store energy and as structures for other biomolecules. Carbon is primarily fixed through photosynthesis, but some organisms use chemosynthesis in the absence of sunlight. Chemosynthesis is carbon fixation driven by chemical energy rather than from sunlight.
The process of biological carbon fixation plays a crucial role in the global carbon cycle, as it serves as the primary mechanism for removing CO2 from the atmosphere and incorporating it into living biomass. The primary production of organic compounds allows carbon to enter the biosphere. Carbon is considered essential for life as a base element for building organic compounds. The element of carbon forms the bases biogeochemical cycles (or nutrient cycles) and drives communities of living organisms. Understanding biological carbon fixation is essential for comprehending ecosystem dynamics, climate regulation, and the sustainability of life on Earth.
Organisms that grow by fixing carbon, such as most plants and algae, are called autotrophs. These include photoautotrophs (which use sunlight) and lithoautotrophs (which use inorganic oxidation). Heterotrophs, such as animals and fungi, are not capable of carbon fixation but are able to grow by consuming the carbon fixed by autotrophs or other heterotrophs.
Seven natural autotrophic carbon fixation pathways are currently known. They are the: i) Calvin-Benson-Bassham (Calvin Cycle), ii) Reverse Krebs (rTCA) cycle, iii) the reductive acetyl-CoA (Wood-Ljungdahl pathway), iv) 3-hydroxy propionate bicycle, v) 3-hydroypropionate/4- hydroxybutyrate (3-HP/4-HB) cycle, vi) the dicarboxylate/ 4-hydroxybutyrate (DC/4-HB) cycle, and vii) the reductive glycine (rGly) pathway. "Fixed carbon," "reduced carbon," and "organic carbon" may all be used interchangeably to refer to various organic compounds.
Net vs. gross CO2 fixation

The primary form of fixed inorganic carbon is carbon dioxide (CO2). It is estimated that approximately 250 billion tons of carbon dioxide are converted by photosynthesis annually. The majority of the fixation occurs in terrestrial environments, especially the tropics. The gross amount of carbon dioxide fixed is much larger since approximately 40% is consumed by respiration following photosynthesis. Historically, it is estimated that approximately 2×10 billion tons of carbon has been fixed since the origin of life.
Overview of the carbon fixation cycles
Seven autotrophic carbon fixation pathways are known: the Calvin Cycle, the Reverse Krebs Cycle, the reductive acetyl-CoA, the 3-HP bicycle, the 3-HP/4-HB cycle, the DC/4-HB cycles, and the reductive glycine pathway.
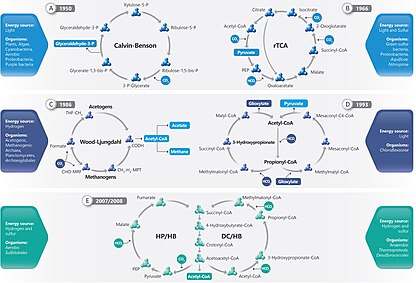
The organisms the Calvin cycle is found in are plants, algae, cyanobacteria, aerobic proteobacteria, and purple bacteria. The Calvin cycle fixes carbon in the chloroplasts of plants and algae, and in the cyanobacteria. It also fixes carbon in the anoxygenic photosynthesis in one type of Pseudomonadota called purple bacteria, and in some non-phototrophic Pseudomonadota.
Of the other autotrophic pathways, three are known only in bacteria (the reductive citric acid cycle, the 3-hydroxypropionate cycle, and the reductive glycine pathway), two only in archaea (two variants of the 3-hydroxypropionate cycle), and one in both bacteria and archaea (the reductive acetyl CoA pathway). Sulfur- and hydrogen-oxidizing bacteria often use the Calvin cycle or the reductive citric acid cycle.
List of pathways

Calvin cycle
The Calvin cycle accounts for 90% of biological carbon fixation. Consuming adenosine triphosphate (ATP) and nicotinamide adenine dinucleotide phosphate (NADPH), the Calvin cycle in plants accounts for the predominance of carbon fixation on land. In algae and cyanobacteria, it accounts for the dominance of carbon fixation in the oceans. The Calvin cycle converts carbon dioxide into sugar, as triose phosphate (TP), which is glyceraldehyde 3-phosphate (GAP) together with dihydroxyacetone phosphate (DHAP):
- 3 CO2 + 12 e + 12 H + Pi → TP + 4 H2O
An alternative perspective accounts for NADPH (source of e) and ATP:
- 3 CO2 + 6 NADPH + 6 H + 9 ATP + 5 H2O → TP + 6 NADP + 9 ADP + 8 Pi
The formula for inorganic phosphate (Pi) is HOPO3 + 2 H.
Formulas for triose and TP are C2H3O2-CH2OH and C2H3O2-CH2OPO3 + 2 H.

Reverse Krebs cycle
The reverse Krebs cycle, also known as the reverse TCA cycle (rTCA) or reductive citric acid cycle, is an alternative to the standard Calvin-Benson cycle for carbon fixation. It has been found in strict anaerobic or microaerobic bacteria (as Aquificales) and anaerobic archea. It was discovered by Evans, Buchanan and Arnon in 1966 working with the photosynthetic green sulfur bacterium Chlorobium limicola. In particular, it is one of the most used pathways in hydrothermal vents by the Campylobacterota. This feature allows primary production in the ocean's aphotic environments, or "dark primary production." Without it, there would be no primary production in aphotic environments, which would lead to habitats without life.
The cycle involves the biosynthesis of acetyl-CoA from two molecules of CO2. The key steps of the reverse Krebs cycle are:
- Oxaloacetate to malate, using NADH + H
- Fumarate to succinate, catalyzed by an oxidoreductase, Fumarate reductase
- Succinate to succinyl-CoA, an ATP-dependent step
- Succinyl-CoA to alpha-ketoglutarate, using one molecule of CO2
- Alpha-ketoglutarate to isocitrate, using NADPH + H and another molecule of CO2
- Citrate converted into oxaloacetate and acetyl-CoA, this is an ATP dependent step and the key enzyme is the ATP citrate lyase
This pathway is cyclic due to the regeneration of the oxaloacetate.
The bacteria Gammaproteobacteria and Riftia pachyptila switch from the Calvin-Benson cycle to the rTCA cycle in response to concentrations of H2S.

Reductive acetyl CoA pathway
The reductive acetyl CoA pathway (CoA) pathway, also known as the Wood-Ljungdahl pathway uses CO2 as electron acceptor and carbon source, and H2 as an electron donor to form acetic acid. This metabolism is widespread within the phylum Bacillota, especially in the Clostridia.
The pathway is also used by methanogens, which are mainly Euryarchaeota, and several anaerobic chemolithoautotrophs, such as sulfate-reducing bacteria and archaea. It is probably performed also by the Brocadiales, an order of Planctomycetota that oxidize ammonia in anaerobic conditions. Hydrogenotrophic methanogenesis, which is only found in certain archaea and accounts for 80% of global methanogenesis, is also based on the reductive acetyl CoA pathway.
The Carbon Monoxide Dehydrogenase/Acetyl-CoA Synthase is the oxygen-sensitive enzyme that permits the reduction of CO2 to CO and the synthesis of acetyl-CoA in several reactions.
One branch of this pathway, the methyl branch, is similar but non-homologous between bacteria and archaea. In this branch happens the reduction of CO2 to a methyl residue bound to a cofactor. The intermediates are formate for bacteria and formyl-methanofuran for archaea, and also the carriers, tetrahydrofolate and tetrahydropterins respectively in bacteria and archaea, are different, such as the enzymes forming the cofactor-bound methyl group.
Otherwise, the carbonyl branch is homologous between the two domains and consists of the reduction of another molecule of CO2 to a carbonyl residue bound to an enzyme, catalyzed by the CO dehydrogenase/acetyl-CoA synthase. This key enzyme is also the catalyst for the formation of acetyl-CoA starting from the products of the previous reactions, the methyl and the carbonyl residues.
This carbon fixation pathway requires only one molecule of ATP for the production of one molecule of pyruvate, which makes this process one of the main choice for chemolithoautotrophs limited in energy and living in anaerobic conditions.
3-Hydroxypropionate bicycle
The 3-hydroxypropionate bicycle, also known as 3-HP/malyl-CoA cycle, discovered only in 1989, is utilized by green non-sulfur phototrophs of Chloroflexaceae family, including the maximum exponent of this family Chloroflexus auranticus by which this way was discovered and demonstrated. The 3-hydroxypropionate bicycle is composed of two cycles, and the name of this way comes from the 3-hydroxypropionate, which corresponds to an intermediate characteristic of it.

The first cycle is a way of synthesis of glyoxylate. During this cycle, two equivalents of bicarbonate are fixed by the action of two enzymes: the acetyl-CoA carboxylase catalyzes the carboxylation of the acetyl-CoA to malonyl-CoA and propionyl-CoA carboxylase catalyses the carboxylation of propionyl-CoA to methylamalonyl-CoA. From this point, a series of reactions lead to the formation of glyoxylate, which will thus become part of the second cycle.
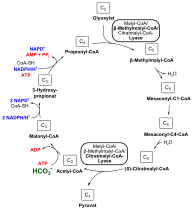
In the second cycle, glyoxylate is approximately one equivalent of propionyl-CoA forming methylamalonyl-CoA. This, in turn, is then converted through a series of reactions into citramalyl-CoA. The citramalyl-CoA is split into pyruvate and acetyl-CoA thanks to the enzyme MMC lyase. The pyruvate is released at this point, while the acetyl-CoA is reused and carboxylated again at malonyl-CoA, thus reconstituting the cycle.
A total of 19 reactions are involved in the 3-hydroxypropionate bicycle, and 13 multifunctional enzymes are used. The multi-functionality of these enzymes is an important feature of this pathway which thus allows the fixation of three bicarbonate molecules.
It is a costly pathway: 7 ATP molecules are consumed to synthesise the new pyruvate and 3 ATP for the phosphate triose.
An important characteristic of this cycle is that it allows the co-assimilation of numerous compounds, making it suitable for the mixotrophic organisms.
Cycles related to the 3-hydroxypropionate cycle
A variant of the 3-hydroxypropionate cycle was found to operate in the aerobic extreme thermoacidophile archaeon Metallosphaera sedula. This pathway is called the 3-hydroxypropionate/4-hydroxybutyrate (3-HP/4-HB) cycle.
Yet another variant of the 3-hydroxypropionate cycle is the dicarboxylate/4-hydroxybutyrate (DC/4-HB) cycle. It was discovered in anaerobic archaea. It was proposed in 2008 for the hyperthermophile archeon Ignicoccus hospitalis.
Enoyl-CoA carboxylases/reductases
CO2 fixation is catalyzed by enoyl-CoA carboxylases/reductases.
Non-autotrophic pathways
Although no heterotrophs use carbon dioxide in biosynthesis, some carbon dioxide is incorporated in their metabolism. Notably pyruvate carboxylase consumes carbon dioxide (as bicarbonate ions) as part of gluconeogenesis, and carbon dioxide is consumed in various anaplerotic reactions.
6-phosphogluconate dehydrogenase catalyzes the reductive carboxylation of ribulose 5-phosphate to 6-phosphogluconate in E. coli under elevated CO2 concentrations.
Carbon isotope discrimination
Some carboxylases, particularly RuBisCO, preferentially bind the lighter carbon stable isotope carbon-12 over the heavier carbon-13. This is known as carbon isotope discrimination and results in carbon-12 to carbon-13 ratios in the plant that are higher than in the free air. Measurement of this isotopic ratio is important in the evaluation of water use efficiency in plants, and also in assessing the possible or likely sources of carbon in global carbon cycle studies.
Biological carbon fixation in soils
In addition to photosynthetic and chemosynthetic processes, biological carbon fixation occurs in soil through the activity of microorganisms, such as bacteria and fungi. These soil microbes play a crucial role in the global carbon cycle by sequestering carbon from decomposed organic matter and recycling it back into the soil, thereby contributing to soil fertility and ecosystem productivity.
In soil environments, organic matter derived from dead plant and animal material undergoes decomposition, a process carried out by a diverse community of microorganisms. During decomposition, complex organic compounds are broken down into simpler molecules by the action of enzymes produced by bacteria, fungi, and other soil organisms. As organic matter is decomposed, carbon is released in various forms, including carbon dioxide (CO2) and dissolved organic carbon (DOC).
However, not all of the carbon released during decomposition is immediately lost to the atmosphere; a significant portion is retained in the soil through processes collectively known as soil carbon sequestration. Soil microbes, particularly bacteria and fungi, play a pivotal role in this process by incorporating decomposed organic carbon into their biomass or by facilitating the formation of stable organic compounds, such as humus and soil organic matter.
One key mechanism by which soil microbes sequester carbon is through the production of microbial biomass. Bacteria and fungi assimilate carbon from decomposed organic matter into their cellular structures as they grow and reproduce. This microbial biomass serves as a reservoir for stored carbon in the soil, effectively sequestering carbon from the atmosphere.
Additionally, soil microbes contribute to the formation of stable soil organic matter through the synthesis of extracellular polymers, enzymes, and other biochemical compounds. These substances help bind together soil particles, forming aggregates that protect organic carbon from microbial decomposition and physical erosion. Over time, these aggregates accumulate in the soil, resulting in the formation of soil organic matter, which can persist for centuries to millennia.
The sequestration of carbon in soil not only helps mitigate the accumulation of atmospheric CO2 and mitigate climate change but also enhances soil fertility, water retention, and nutrient cycling, thereby supporting plant growth and ecosystem productivity. Consequently, understanding the role of soil microbes in biological carbon fixation is essential for managing soil health, mitigating climate change, and promoting sustainable land management practices.
Biological carbon fixation is a fundamental process that sustains life on Earth by regulating atmospheric CO2 levels, supporting the growth of plants and other photosynthetic organisms, and maintaining ecological balance.
See also
References
- ^ Santos Correa S, Schultz J, Lauersen KJ, Soares Rosado A (1 May 2023). "Natural carbon fixation and advances in synthetic engineering for redesigning and creating new fixation pathways". Journal of Advanced Research. 47: 75–92. doi:10.1016/j.jare.2022.07.011. hdl:10754/680126. ISSN 2090-1232. PMC 10173188. PMID 35918056.
- ^ Santos Correa S, Schultz J, Lauersen KJ, Soares Rosado A (1 May 2023). "Natural carbon fixation and advances in synthetic engineering for redesigning and creating new fixation pathways". Journal of Advanced Research. 47: 75–92. doi:10.1016/j.jare.2022.07.011. hdl:10754/680126. ISSN 2090-1232. PMC 10173188. PMID 35918056.
- ^ Berg JM, Tymoczko JL, Stryer L (2013). Stryer Biochemie. doi:10.1007/978-3-8274-2989-6. ISBN 978-3-8274-2988-9.
- Bierbaumer S, Nattermann M, Schulz L, Zschoche R, Erb T, Winkler C, et al. (24 January 2023). "Enzymatic Conversion of CO2: From Natural to Artificial Utilization". Chemical Reviews. doi:10.1021/acs.chemrev.2c00581. PMC 10176493.
- Geider RJ, et al. (2001). "Primary productivity of planet earth: biological determinants and physical constraints in terrestrial and aquatic habitats". Global Change Biology. 7 (8): 849–882. Bibcode:2001GCBio...7..849G. doi:10.1046/j.1365-2486.2001.00448.x. S2CID 41335311.
- Geider RJ, et al. (2001). "Primary productivity of planet earth: biological determinants and physical constraints in terrestrial and aquatic habitats". Global Change Biology. 7 (8): 849–882. Bibcode:2001GCBio...7..849G. doi:10.1046/j.1365-2486.2001.00448.x. S2CID 41335311.
- Raghavendra, A. S. (2003-01-01), Thomas, Brian (ed.), "Photosynthesis and Partitioning | C3 Plants", Encyclopedia of Applied Plant Sciences, Oxford: Elsevier, pp. 673–680, ISBN 978-0-12-227050-5, retrieved 2021-03-21
- Crockford PW, Bar On YM, Ward LM, Milo R, Halevy I (November 2023). "The geologic history of primary productivity". Current Biology. 33 (21): 4741–4750.e5. Bibcode:2023CBio...33E4741C. doi:10.1016/j.cub.2023.09.040. ISSN 0960-9822. PMID 37827153. S2CID 263839383.
- Santos Correa S, Schultz J, Lauersen KJ, Soares Rosado A (1 May 2023). "Natural carbon fixation and advances in synthetic engineering for redesigning and creating new fixation pathways". Journal of Advanced Research. 47: 75–92. doi:10.1016/j.jare.2022.07.011. hdl:10754/680126. ISSN 2090-1232. PMC 10173188. PMID 35918056.
- Swan BK, Martinez-Garcia M, Preston CM, Sczyrba A, Woyke T, Lamy D, et al. (September 2011). "Potential for chemolithoautotrophy among ubiquitous bacteria lineages in the dark ocean". Science. 333 (6047): 1296–300. Bibcode:2011Sci...333.1296S. doi:10.1126/science.1203690. PMID 21885783. S2CID 206533092.
- Encyclopedia of Microbiology. Academic Press. 2009. pp. 83–84. ISBN 978-0-12-373944-5.
- Raines CA (1 January 2003). "The Calvin cycle revisited". Photosynthesis Research. 75 (1): 1–10. doi:10.1023/A:1022421515027. ISSN 1573-5079. PMID 16245089.
- Fuchs G (13 October 2011). "Alternative pathways of carbon dioxide fixation: insights into the early evolution of life?". Annual Review of Microbiology. 65 (1): 631–58. doi:10.1146/annurev-micro-090110-102801. PMID 21740227.
- Grzymski JJ, Murray AE, Campbell BJ, Kaplarevic M, Gao GR, Lee C, et al. (November 2008). "Metagenome analysis of an extreme microbial symbiosis reveals eurythermal adaptation and metabolic flexibility". Proceedings of the National Academy of Sciences of the United States of America. 105 (45): 17516–21. Bibcode:2008PNAS..10517516G. doi:10.1073/pnas.0802782105. PMC 2579889. PMID 18987310.
- Baltar F, Herndl GJ (11 June 2019). "Is dark carbon fixation relevant for oceanic primary production estimates?" (PDF). Biogeosciences. doi:10.5194/bg-2019-223.
- ^ Hügler M, Sievert SM (15 January 2011). "Beyond the Calvin cycle: autotrophic carbon fixation in the ocean". Annual Review of Marine Science. 3 (1): 261–89. Bibcode:2011ARMS....3..261H. doi:10.1146/annurev-marine-120709-142712. PMID 21329206. S2CID 44800487.
- Buchanan BB, Arnon DI (April 1990). "A reverse Krebs cycle in photosynthesis: consensus at last". Photosynthesis Research. 24 (1): 47–53. Bibcode:1990PhoRe..24...47B. doi:10.1007/bf00032643. PMID 24419764. S2CID 2753977.
- Markert S, Arndt C, Felbeck H, Becher D, Sievert SM, Hügler M, et al. (January 2007). "Physiological proteomics of the uncultured endosymbiont of Riftia pachyptila". Science. 315 (5809): 247–50. Bibcode:2007Sci...315..247M. doi:10.1126/science.1132913. hdl:1912/1514. OCLC 655249163. PMID 17218528. S2CID 45745396.
- Ljungdahl LG (2009). "A life with acetogens, thermophiles, and cellulolytic anaerobes". Annual Review of Microbiology. 63 (1): 1–25. doi:10.1146/annurev.micro.091208.073617. PMID 19575555.
- ^ Drake HL, Gössner AS, Daniel SL (March 2008). "Old acetogens, new light". Annals of the New York Academy of Sciences. 1125 (1): 100–28. Bibcode:2008NYASA1125..100D. doi:10.1196/annals.1419.016. PMID 18378590. S2CID 24050060.
- Strous M, Pelletier E, Mangenot S, Rattei T, Lehner A, Taylor MW, et al. (April 2006). "Deciphering the evolution and metabolism of an anammox bacterium from a community genome". Nature. 440 (7085): 790–4. Bibcode:2006Natur.440..790S. doi:10.1038/nature04647. hdl:2066/35981. PMID 16598256. S2CID 4402553.
- ^ Pezacka E, Wood HG (October 1984). "Role of carbon monoxide dehydrogenase in the autotrophic pathway used by acetogenic bacteria". Proceedings of the National Academy of Sciences of the United States of America. 81 (20): 6261–5. Bibcode:1984PNAS...81.6261P. doi:10.1073/pnas.81.20.6261. PMC 391903. PMID 6436811.
- Strauss G, Fuchs G (August 1993). "Enzymes of a novel autotrophic CO2 fixation pathway in the phototrophic bacterium Chloroflexus aurantiacus, the 3-hydroxypropionate cycle". European Journal of Biochemistry. 215 (3): 633–43. doi:10.1111/j.1432-1033.1993.tb18074.x. PMID 8354269.
- Herter S, Busch A, Fuchs G (November 2002). "L-Malyl-coenzyme A lyase/beta-methylmalyl-coenzyme A lyase from Chloroflexus aurantiacus, a bifunctional enzyme involved in autotrophic CO2 fixation". Journal of Bacteriology. 184 (21): 5999–6006. doi:10.1128/jb.184.21.5999-6006.2002. PMC 135395. PMID 12374834.
- ^ Berg IA (March 2011). "Ecological aspects of the distribution of different autotrophic CO2 fixation pathways". Applied and Environmental Microbiology. 77 (6): 1925–36. Bibcode:2011ApEnM..77.1925B. doi:10.1128/aem.02473-10. PMC 3067309. PMID 21216907.
- ^ Zarzycki J, Brecht V, Müller M, Fuchs G (December 2009). "Identifying the missing steps of the autotrophic 3-hydroxypropionate CO2 fixation cycle in Chloroflexus aurantiacus". Proceedings of the National Academy of Sciences of the United States of America. 106 (50): 21317–22. doi:10.1073/pnas.0908356106. PMC 2795484. PMID 19955419.
- Berg IA, Kockelkorn D, Buckel W, Fuchs G (December 2007). "A 3-hydroxypropionate/4-hydroxybutyrate autotrophic carbon dioxide assimilation pathway in Archaea". Science. 318 (5857): 1782–6. Bibcode:2007Sci...318.1782B. doi:10.1126/science.1149976. PMID 18079405. S2CID 13218676.
- Huber H, Gallenberger M, Jahn U, Eylert E, Berg IA, Kockelkorn D, et al. (June 2008). "A dicarboxylate/4-hydroxybutyrate autotrophic carbon assimilation cycle in the hyperthermophilic Archaeum Ignicoccus hospitalis". Proceedings of the National Academy of Sciences of the United States of America. 105 (22): 7851–6. Bibcode:2008PNAS..105.7851H. doi:10.1073/pnas.0801043105. PMC 2409403. PMID 18511565.
- Schwander T, Schada von Borzyskowski L, Burgener S, Cortina NS, Erb TJ (2016). "A synthetic pathway for the fixation of carbon dioxide in vitro". Science. 354 (6314): 900–904. Bibcode:2016Sci...354..900S. doi:10.1126/science.aah5237. PMC 5892708. PMID 27856910.
- Nicole Kresge, Robert D. Simoni, Robert L. Hill (2005). "The Discovery of Heterotrophic Carbon Dioxide Fixation by Harland G. Wood". The Journal of Biological Chemistry. 280 (18): e15.
- Satanowski A, Dronsella B, Noor E, Vögeli B, He H, Wichmann P, et al. (November 2020). "Awakening a latent carbon fixation cycle in Escherichia coli". Nature Communications. 11 (1): 5812. Bibcode:2020NatCo..11.5812S. doi:10.1038/s41467-020-19564-5. PMC 7669889. PMID 33199707.
- Adiredjo AL, Navaud O, Muños S, Langlade NB, Lamaze T, Grieu P (3 July 2014). "Genetic control of water use efficiency and leaf carbon isotope discrimination in sunflower (Helianthus annuus L.) subjected to two drought scenarios". PLOS ONE. 9 (7): e101218. Bibcode:2014PLoSO...9j1218A. doi:10.1371/journal.pone.0101218. PMC 4081578. PMID 24992022.
- Farquhar GD, Ehleringer JR, Hubick KT (June 1989). "Carbon Isotope Discrimination and Photosynthesis". Annual Review of Plant Physiology and Plant Molecular Biology. 40 (1): 503–537. doi:10.1146/annurev.pp.40.060189.002443. S2CID 12988287.
- Seibt U, Rajabi A, Griffiths H, Berry JA (March 2008). "Carbon isotopes and water use efficiency: sense and sensitivity". Oecologia. 155 (3): 441–54. Bibcode:2008Oecol.155..441S. doi:10.1007/s00442-007-0932-7. PMID 18224341. S2CID 451126.
- LibreTexts Biology, 3.4: Biochemical compounds
- Soil Science Society of America (SSSA). Physical Properties of Soil – Soil Texture
Further reading
- Keeling PJ (October 2004). "Diversity and evolutionary history of plastids and their hosts". American Journal of Botany. 91 (10): 1481–93. doi:10.3732/ajb.91.10.1481. PMID 21652304. S2CID 17522125.
- Keeling PJ (2009). "Chromalveolates and the evolution of plastids by secondary endosymbiosis" (PDF). The Journal of Eukaryotic Microbiology. 56 (1): 1–8. doi:10.1111/j.1550-7408.2008.00371.x. PMID 19335769. S2CID 34259721. Archived from the original (PDF) on 9 July 2009.
- Keeling PJ (March 2010). "The endosymbiotic origin, diversification and fate of plastids". Philosophical Transactions of the Royal Society of London. Series B, Biological Sciences. 365 (1541): 729–48. doi:10.1098/rstb.2009.0103. PMC 2817223. PMID 20124341.
- Price DC, Chan CX, Yoon HS, Yang EC, Qiu H, Weber AP, et al. (February 2012). "Cyanophora paradoxa genome elucidates origin of photosynthesis in algae and plants" (PDF). Science. 335 (6070): 843–7. Bibcode:2012Sci...335..843P. doi:10.1126/science.1213561. PMID 22344442. S2CID 17190180. Archived from the original (PDF) on 14 May 2013.
- Spiegel FW (February 2012). "Evolution. Contemplating the first Plantae". Science. 335 (6070): 809–10. Bibcode:2012Sci...335..809S. doi:10.1126/science.1218515. PMID 22344435. S2CID 36584136.
- Timme RE, Bachvaroff TR, Delwiche CF (2012). "Broad phylogenomic sampling and the sister lineage of land plants". PLOS ONE. 7 (1): e29696. Bibcode:2012PLoSO...729696T. doi:10.1371/journal.pone.0029696. PMC 3258253. PMID 22253761.
Metabolism, catabolism, anabolism | |||||||||||||||||||||||||||||||||
---|---|---|---|---|---|---|---|---|---|---|---|---|---|---|---|---|---|---|---|---|---|---|---|---|---|---|---|---|---|---|---|---|---|
General | |||||||||||||||||||||||||||||||||
Energy metabolism |
| ||||||||||||||||||||||||||||||||
Specific paths |
|